A | B | C | D | E | F | G | H | CH | I | J | K | L | M | N | O | P | Q | R | S | T | U | V | W | X | Y | Z | 0 | 1 | 2 | 3 | 4 | 5 | 6 | 7 | 8 | 9

Nuclear weapon designs are physical, chemical, and engineering arrangements that cause the physics package[1] of a nuclear weapon to detonate. There are three existing basic design types:
- pure fission weapons are the simplest, least technically demanding, were the first nuclear weapons built, and so far the only type ever used in warfare, by the United States on Japan in World War II
- boosted fission weapons increase yield beyond that of the implosion design, by using small quantities of fusion fuel to enhance the fission chain reaction. Boosting can more than double the weapon's fission energy yield.
- staged thermonuclear weapons are arrangements of two or more "stages", most usually two. The first stage is normally a boosted fission weapon as above (except for the earliest thermonuclear weapons, which used a pure fission weapon instead). Its detonation causes it to shine intensely with x-radiation, which illuminates and implodes the second stage filled with a large quantity of fusion fuel. This sets in motion a sequence of events which results in a thermonuclear, or fusion, burn. This process affords potential yields up to hundreds of times those of fission weapons.[2]
Pure fission weapons have been the first type to be built by new nuclear powers. Large industrial states with well-developed nuclear arsenals have two-stage thermonuclear weapons, which are the most compact, scalable, and cost effective option, once the necessary technical base and industrial infrastructure are built.
Most known innovations in nuclear weapon design originated in the United States, though some were later developed independently by other states.[3]
In early news accounts, pure fission weapons were called atomic bombs or A-bombs and weapons involving fusion were called hydrogen bombs or H-bombs. Practitioners of nuclear policy, however, favor the terms nuclear and thermonuclear, respectively.
Nuclear weapons |
---|
![]() |
Background |
Nuclear-armed states |
|
Nuclear reactions
Nuclear fission separates or splits heavier atoms to form lighter atoms. Nuclear fusion combines lighter atoms to form heavier atoms. Both reactions generate roughly a million times more energy than comparable chemical reactions, making nuclear bombs a million times more powerful than non-nuclear bombs, which a French patent claimed in May 1939.[4]
In some ways, fission and fusion are opposite and complementary reactions, but the particulars are unique for each. To understand how nuclear weapons are designed, it is useful to know the important similarities and differences between fission and fusion. The following explanation uses rounded numbers and approximations.[5]
Fission
When a free neutron hits the nucleus of a fissile atom like uranium-235 (235U), the uranium nucleus splits into two smaller nuclei called fission fragments, plus more neutrons (for 235U three about as often as two; an average of just under 2.5 per fission). The fission chain reaction in a supercritical mass of fuel can be self-sustaining because it produces enough surplus neutrons to offset losses of neutrons escaping the supercritical assembly. Most of these have the speed (kinetic energy) required to cause new fissions in neighboring uranium nuclei.[6]
The uranium-235 nucleus can split in many ways, provided the charge numbers add up to 92 and the mass numbers add up to 236 (uranium-235 plus the neutron that caused the split). The following equation shows one possible split, namely into strontium-95 (95Sr), xenon-139 (139Xe), and two neutrons (n), plus energy:[7]
The immediate energy release per atom is about 180 million electron volts (MeV); i.e., 74 TJ/kg. Only 7% of this is gamma radiation and kinetic energy of fission neutrons. The remaining 93% is kinetic energy (or energy of motion) of the charged fission fragments, flying away from each other mutually repelled by the positive charge of their protons (38 for strontium, 54 for xenon). This initial kinetic energy is 67 TJ/kg, imparting an initial speed of about 12,000 kilometers per second. The charged fragments' high electric charge causes many inelastic coulomb collisions with nearby nuclei, and these fragments remain trapped inside the bomb's fissile pit and tamper until their motion is converted into heat. Given the speed of the fragments and the mean free path between nuclei in the compressed fuel assembly (for the implosion design), this takes about a millionth of a second (a microsecond), by which time the core and tamper of the bomb have expanded to plasma several meters in diameter with a temperature of tens of millions of degrees Celsius.
This is hot enough to emit black-body radiation in the X-ray spectrum. These X-rays are absorbed by the surrounding air, producing the fireball and blast of a nuclear explosion.
Most fission products have too many neutrons to be stable so they are radioactive by beta decay, converting neutrons into protons by throwing off beta particles (electrons) and gamma rays. Their half lives range from milliseconds to about 200,000 years. Many decay into isotopes that are themselves radioactive, so from 1 to 6 (average 3) decays may be required to reach stability.[8] In reactors, the radioactive products are the nuclear waste in spent fuel. In bombs, they become radioactive fallout, both local and global.[9]
Meanwhile, inside the exploding bomb, the free neutrons released by fission carry away about 3% of the initial fission energy. Neutron kinetic energy adds to the blast energy of a bomb, but not as effectively as the energy from charged fragments, since neutrons do not give up their kinetic energy as quickly in collisions with charged nuclei or electrons. The dominant contribution of fission neutrons to the bomb's power is the initiation of subsequent fissions. Over half of the neutrons escape the bomb core, but the rest strike 235U nuclei causing them to fission in an exponentially growing chain reaction (1, 2, 4, 8, 16, etc.). Starting from one atom, the number of fissions can theoretically double a hundred times in a microsecond, which could consume all uranium or plutonium up to hundreds of tons by the hundredth link in the chain. Typically in a modern weapon, the weapon's pit contains 3.5 to 4.5 kilograms (7.7 to 9.9 lb) of plutonium and at detonation produces approximately 5 to 10 kilotonnes of TNT (21 to 42 TJ) yield, representing the fissioning of approximately 0.5 kilograms (1.1 lb) of plutonium.[10][11]
Materials which can sustain a chain reaction are called fissile. The two fissile materials used in nuclear weapons are: 235U, also known as highly enriched uranium (HEU), "oralloy" meaning "Oak Ridge alloy",[12] or "25" (a combination of the last digit of the atomic number of uranium-235, which is 92, and the last digit of its mass number, which is 235); and 239Pu, also known as plutonium-239, or "49" (from "94" and "239").[13]
Uranium's most common isotope, 238U, is fissionable but not fissile, meaning that it cannot sustain a chain reaction because its daughter fission neutrons are not (on average) energetic enough to cause follow-on 238U fissions. However, the neutrons released by fusion of the heavy hydrogen isotopes deuterium and tritium will fission 238U. This 238U fission reaction in the outer jacket of the secondary assembly of a two-stage thermonuclear bomb produces by far the greatest fraction of the bomb's energy yield, as well as most of its radioactive debris.
For national powers engaged in a nuclear arms race, this fact of 238U's ability to fast-fission from thermonuclear neutron bombardment is of central importance. The plenitude and cheapness of both bulk dry fusion fuel (lithium deuteride) and 238U (a byproduct of uranium enrichment) permit the economical production of very large nuclear arsenals, in comparison to pure fission weapons requiring the expensive 235U or 239Pu fuels.
Fusion
Fusion produces neutrons which dissipate energy from the reaction.[14] In weapons, the most important fusion reaction is called the D-T reaction. Using the heat and pressure of fission, hydrogen-2, or deuterium (2D), fuses with hydrogen-3, or tritium (3T), to form helium-4 (4He) plus one neutron (n) and energy:[15]

The total energy output, 17.6 MeV, is one tenth of that with fission, but the ingredients are only one-fiftieth as massive, so the energy output per unit mass is approximately five times as great. In this fusion reaction, 14 of the 17.6 MeV (80% of the energy released in the reaction) shows up as the kinetic energy of the neutron, which, having no electric charge and being almost as massive as the hydrogen nuclei that created it, can escape the scene without leaving its energy behind to help sustain the reaction – or to generate x-rays for blast and fire.[citation needed]
The only practical way to capture most of the fusion energy is to trap the neutrons inside a massive bottle of heavy material such as lead, uranium, or plutonium. If the 14 MeV neutron is captured by uranium (of either isotope; 14 MeV is high enough to fission both 235U and 238U) or plutonium, the result is fission and the release of 180 MeV of fission energy, multiplying the energy output tenfold.[citation needed]
For weapon use, fission is necessary to start fusion, helps to sustain fusion, and captures and multiplies the energy carried by the fusion neutrons. In the case of a neutron bomb (see below), the last-mentioned factor does not apply, since the objective is to facilitate the escape of neutrons, rather than to use them to increase the weapon's raw power.[citation needed]
Tritium production
An essential nuclear reaction is the one that creates tritium, or hydrogen-3. Tritium is employed in two ways. First, pure tritium gas is produced for placement inside the cores of boosted fission devices in order to increase their energy yields. This is especially so for the fission primaries of thermonuclear weapons. The second way is indirect, and takes advantage of the fact that the neutrons emitted by a supercritical fission "spark plug" in the secondary assembly of a two-stage thermonuclear bomb will produce tritium in situ when these neutrons collide with the lithium nuclei in the bomb's lithium deuteride fuel supply.
Elemental gaseous tritium for fission primaries is also made by bombarding lithium-6 (6Li) with neutrons (n), only in a nuclear reactor. This neutron bombardment will cause the lithium-6 nucleus to split, producing an alpha particle, or helium-4 (4He), plus a triton (3T) and energy:[15]
The neutrons are supplied by the nuclear reactor in a way similar to production of plutonium 239Pu from 238U feedstock: target rods of the 6Li feedstock are arranged around a uranium-fueled core, and are removed for processing once it has been calculated that most of the lithium nuclei have been transmuted to tritium.
Of the four basic types of nuclear weapon, the first, pure fission, uses the first of the three nuclear reactions above. The second, fusion-boosted fission, uses the first two. The third, two-stage thermonuclear, uses all three.
Pure fission weapons

The first task of a nuclear weapon design is to rapidly assemble a supercritical mass of fissile (weapon grade) uranium or plutonium. A supercritical mass is one in which the percentage of fission-produced neutrons captured by other neighboring fissile nuclei is large enough that each fission event, on average, causes more than one follow-on fission event. Neutrons released by the first fission events induce subsequent fission events at an exponentially accelerating rate. Each follow-on fissioning continues a sequence of these reactions that works its way throughout the supercritical mass of fuel nuclei. This process is conceived and described colloquially as the nuclear chain reaction.
To start the chain reaction in a supercritical assembly, at least one free neutron must be injected and collide with a fissile fuel nucleus. The neutron joins with the nucleus (technically a fusion event) and destabilizes the nucleus, which explodes into two middleweight nuclear fragments (from the severing of the strong nuclear force holding the mutually-repulsive protons together), plus two or three free neutrons. These race away and collide with neighboring fuel nuclei. This process repeats over and over until the fuel assembly goes subcritical (from thermal expansion), after which the chain reaction shuts down because the daughter neutrons can no longer find new fuel nuclei to hit before escaping the less-dense fuel mass. Each following fission event in the chain approximately doubles the neutron population (net, after losses due to some neutrons escaping the fuel mass, and others that collide with any non-fuel impurity nuclei present).
For the gun assembly method (see below) of supercritical mass formation, the fuel itself can be relied upon to initiate the chain reaction. This is because even the best weapon-grade uranium contains a significant number of 238U nuclei. These are susceptible to spontaneous fission events, which occur randomly (it is a quantum mechanical phenomenon). Because the fissile material in a gun-assembled critical mass is not compressed, the design need only ensure the two subcritical masses remain close enough to each other long enough that a 238U spontaneous fission will occur while the weapon is in the vicinity of the target. This is not difficult to arrange as it takes but a second or two in a typical-size fuel mass for this to occur. (Still, many such bombs meant for delivery by air (gravity bomb, artillery shell or rocket) use injected neutrons to gain finer control over the exact detonation altitude, important for the destructive effectiveness of airbursts.)
This condition of spontaneous fission highlights the necessity to assemble the supercritical mass of fuel very rapidly. The time required to accomplish this is called the weapon's critical insertion time. If spontaneous fission were to occur when the supercritical mass was only partially assembled, the chain reaction would begin prematurely. Neutron losses through the void between the two subcritical masses (gun assembly) or the voids between not-fully-compressed fuel nuclei (implosion assembly) would sap the bomb of the number of fission events needed to attain the full design yield. Additionally, heat resulting from the fissions that do occur would work against the continued assembly of the supercritical mass, from thermal expansion of the fuel. This failure is called predetonation. The resulting explosion would be called a "fizzle" by bomb engineers and weapon users. Plutonium's high rate of spontaneous fission makes uranium fuel a necessity for gun-assembled bombs, with their much greater insertion time and much greater mass of fuel required (because of the lack of fuel compression).
There is another source of free neutrons that can spoil a fission explosion. All uranium and plutonium nuclei have a decay mode that results in energetic alpha particles. If the fuel mass contains impurity elements of low atomic number (Z), these charged alphas can penetrate the coulomb barrier of these impurity nuclei and undergo a reaction that yields a free neutron. The rate of alpha emission of fissile nuclei is one to two million times that of spontaneous fission, so weapon engineers are careful to use fuel of high purity.
Fission weapons used in the vicinity of other nuclear explosions must be protected from the intrusion of free neutrons from outside. Such shielding material will almost always be penetrated, however, if the outside neutron flux is intense enough. When a weapon misfires or fizzles because of the effects of other nuclear detonations, it is called nuclear fratricide.
For the implosion-assembled design, once the critical mass is assembled to maximum density, a burst of neutrons must be supplied to start the chain reaction. Early weapons used a modulated neutron generator codenamed "Urchin" inside the pit containing polonium-210 and beryllium separated by a thin barrier. Implosion of the pit crushes the neutron generator, mixing the two metals, thereby allowing alpha particles from the polonium to interact with beryllium to produce free neutrons. In modern weapons, the neutron generator is a high-voltage vacuum tube containing a particle accelerator which bombards a deuterium/tritium-metal hydride target with deuterium and tritium ions. The resulting small-scale fusion produces neutrons at a protected location outside the physics package, from which they penetrate the pit. This method allows better timing of the first fission events in the chain reaction, which optimally should occur at the point of maximum compression/supercriticality. Timing of the neutron injection is a more important parameter than the number of neutrons injected: the first generations of the chain reaction are vastly more effective due to the exponential function by which neutron multiplication evolves.
The critical mass of an uncompressed sphere of bare metal is 50 kg (110 lb) for uranium-235 and 16 kg (35 lb) for delta-phase plutonium-239. In practical applications, the amount of material required for criticality is modified by shape, purity, density, and the proximity to neutron-reflecting material, all of which affect the escape or capture of neutrons.
To avoid a premature chain reaction during handling, the fissile material in the weapon must be kept subcritical. It may consist of one or more components containing less than one uncompressed critical mass each. A thin hollow shell can have more than the bare-sphere critical mass, as can a cylinder, which can be arbitrarily long without ever reaching criticality. Another method of reducing criticality risk is to incorporate material with a large cross-section for neutron capture, such as boron (specifically 10B comprising 20% of natural boron). Naturally this neutron absorber must be removed before the weapon is detonated. This is easy for a gun-assembled bomb: the projectile mass simply shoves the absorber out of the void between the two subcritical masses by the force of its motion.
The use of plutonium affects weapon design due to its high rate of alpha emission. This results in Pu metal spontaneously producing significant heat; a 5 kilogram mass-produces 9.68 watts of thermal power. Such a piece would feel warm to the touch, which is no problem if that heat is dissipated promptly and not allowed to build up the temperature. But this is a problem inside a nuclear bomb. For this reason bombs using Pu fuel use aluminum parts to wick away the excess heat, and this complicates bomb design because Al plays no active role in the explosion processes.
A tamper is an optional layer of dense material surrounding the fissile material. Due to its inertia it delays the thermal expansion of the fissioning fuel mass, keeping it supercritical for longer. Often[when?] the same layer serves both as tamper and as neutron reflector.
Gun-type assembly

Little Boy, the Hiroshima bomb, used 64 kg (141 lb) of uranium with an average enrichment of around 80%, or 51 kg (112 lb) of uranium-235, just about the bare-metal critical mass . When assembled inside its tamper/reflector of tungsten carbide, the 64 kg (141 lb) was more than twice critical mass. Before the detonation, the uranium-235 was formed into two sub-critical pieces, one of which was later fired down a gun barrel to join the other, starting the nuclear explosion. Analysis shows that less than 2% of the uranium mass underwent fission;[16] the remainder, representing most of the entire wartime output of the giant Y-12 factories at Oak Ridge, scattered uselessly.[17]
The inefficiency was caused by the speed with which the uncompressed fissioning uranium expanded and became sub-critical by virtue of decreased density. Despite its inefficiency, this design, because of its shape, was adapted for use in small-diameter, cylindrical artillery shells (a gun-type warhead fired from the barrel of a much larger gun).[citation needed] Such warheads were deployed by the United States until 1992, accounting for a significant fraction of the 235U in the arsenal[citation needed], and were some of the first weapons dismantled to comply with treaties limiting warhead numbers.[citation needed] The rationale for this decision was undoubtedly a combination of the lower yield and grave safety issues associated with the gun-type design.[citation needed]
Implosion-type

For both the Trinity device and the Fat Man (Nagasaki) bomb, nearly identical plutonium fission through implosion designs were used. The Fat Man device specifically used 6.2 kg (14 lb), about 350 ml or 12 US fl oz in volume, of Pu-239, which is only 41% of bare-sphere critical mass . Surrounded by a U-238 reflector/tamper, the Fat Man's pit was brought close to critical mass by the neutron-reflecting properties of the U-238. During detonation, criticality was achieved by implosion. The plutonium pit was squeezed to increase its density by simultaneous detonation, as with the "Trinity" test detonation three weeks earlier, of the conventional explosives placed uniformly around the pit. The explosives were detonated by multiple exploding-bridgewire detonators. It is estimated that only about 20% of the plutonium underwent fission; the rest, about 5 kg (11 lb), was scattered.


An implosion shock wave might be of such short duration that only part of the pit is compressed at any instant as the wave passes through it. To prevent this, a pusher shell may be needed. The pusher is located between the explosive lens and the tamper. It works by reflecting some of the shock wave backward, thereby having the effect of lengthening its duration. It is made out of a low density metal – such as aluminium, beryllium, or an alloy of the two metals (aluminium is easier and safer to shape, and is two orders of magnitude cheaper; beryllium has high neutron-reflective capability). Fat Man used an aluminium pusher.
The series of RaLa Experiment tests of implosion-type fission weapon design concepts, carried out from July 1944 through February 1945 at the Los Alamos Laboratory and a remote site 14.3 km (8.9 mi) east of it in Bayo Canyon, proved the practicality of the implosion design for a fission device, with the February 1945 tests positively determining its usability for the final Trinity/Fat Man plutonium implosion design.[18]
The key to Fat Man's greater efficiency was the inward momentum of the massive U-238 tamper. (The natural uranium tamper did not undergo fission from thermal neutrons, but did contribute perhaps 20% of the total yield from fission by fast neutrons). After the chain reaction started in the plutonium, it continued until the explosion reversed the momentum of the implosion and expanded enough to stop the chain reaction. By holding everything together for a few hundred nanoseconds more, the tamper increased the efficiency.
Plutonium pit

The core of an implosion weapon – the fissile material and any reflector or tamper bonded to it – is known as the pit. Some weapons tested during the 1950s used pits made with U-235 alone, or in composite with plutonium,[19] but all-plutonium pits are the smallest in diameter and have been the standard since the early 1960s.[citation needed]
Casting and then machining plutonium is difficult not only because of its toxicity, but also because plutonium has many different metallic phases. As plutonium cools, changes in phase result in distortion and cracking. This distortion is normally overcome by alloying it with 30–35 mMol (0.9–1.0% by weight) gallium, forming a plutonium-gallium alloy, which causes it to take up its delta phase over a wide temperature range.[20] When cooling from molten it then has only a single phase change, from epsilon to delta, instead of the four changes it would otherwise pass through. Other trivalent metals would also work, but gallium has a small neutron absorption cross section and helps protect the plutonium against corrosion. A drawback is that gallium compounds are corrosive and so if the plutonium is recovered from dismantled weapons for conversion to plutonium dioxide for power reactors, there is the difficulty of removing the gallium.[citation needed]
Because plutonium is chemically reactive it is common to plate the completed pit with a thin layer of inert metal, which also reduces the toxic hazard.[21] The gadget used galvanic silver plating; afterward, nickel deposited from nickel tetracarbonyl vapors was used,[21] but thereafter and since, gold became the preferred material.[citation needed] Recent designs improve safety by plating pits with vanadium to make the pits more fire-resistant.[citation needed]
Levitated-pit implosion

The first improvement on the Fat Man design was to put an air space between the tamper and the pit to create a hammer-on-nail impact. The pit, supported on a hollow cone inside the tamper cavity, was said to be "levitated". The three tests of Operation Sandstone, in 1948, used Fat Man designs with levitated pits. The largest yield was 49 kilotons, more than twice the yield of the unlevitated Fat Man.[22]
It was immediately clear[according to whom?] that implosion was the best design for a fission weapon. Its only drawback seemed to be its diameter. Fat Man was 1.5 metres (5 ft) wide vs 61 centimetres (2 ft) for Little Boy.
The Pu-239 pit of Fat Man was only 9.1 centimetres (3.6 in) in diameter, the size of a softball. The bulk of Fat Man's girth was the implosion mechanism, namely concentric layers of U-238, aluminium, and high explosives. The key to reducing that girth was the two-point implosion design.[citation needed]
Two-point linear implosion

In the two-point linear implosion, the nuclear fuel is cast into a solid shape and placed within the center of a cylinder of high explosive. Detonators are placed at either end of the explosive cylinder, and a plate-like insert, or shaper, is placed in the explosive just inside the detonators. When the detonators are fired, the initial detonation is trapped between the shaper and the end of the cylinder, causing it to travel out to the edges of the shaper where it is diffracted around the edges into the main mass of explosive. This causes the detonation to form into a ring that proceeds inward from the shaper.[23]
Due to the lack of a tamper or lenses to shape the progression, the detonation does not reach the pit in a spherical shape. To produce the desired spherical implosion, the fissile material itself is shaped to produce the same effect. Due to the physics of the shock wave propagation within the explosive mass, this requires the pit to be a prolate spheroid, that is, roughly egg shaped. The shock wave first reaches the pit at its tips, driving them inward and causing the mass to become spherical. The shock may also change plutonium from delta to alpha phase, increasing its density by 23%, but without the inward momentum of a true implosion.[citation needed]
The lack of compression makes such designs inefficient, but the simplicity and small diameter make it suitable for use in artillery shells and atomic demolition munitions – ADMs – also known as backpack or suitcase nukes; an example is the W48 artillery shell, the smallest nuclear weapon ever built or deployed. All such low-yield battlefield weapons, whether gun-type U-235 designs or linear implosion Pu-239 designs, pay a high price in fissile material in order to achieve diameters between six and ten inches (15 and 25 cm).[citation needed]
Hollow-pit implosion
A more efficient implosion system uses a hollow pit.[citation needed]
A hollow plutonium pit was the original plan for the 1945 Fat Man bomb, but there was not enough time to develop and test the implosion system for it. A simpler solid-pit design was considered more reliable, given the time constraints, but it required a heavy U-238 tamper, a thick aluminium pusher, and three tons of high explosives.[citation needed]
After the war, interest in the hollow pit design was revived. Its obvious advantage is that a hollow shell of plutonium, shock-deformed and driven inward toward its empty center, would carry momentum into its violent assembly as a solid sphere. It would be self-tamping, requiring a smaller U-238 tamper, no aluminium pusher, and less high explosive.[citation needed]
Fusion-boosted fission

The next step in miniaturization was to speed up the fissioning of the pit to reduce the minimum inertial confinement time. This would allow the efficient fission of the fuel with less mass in the form of tamper or the fuel itself. The key to achieving faster fission would be to introduce more neutrons, and among the many ways to do this, adding a fusion reaction was relatively easy in the case of a hollow pit.[citation needed]
The easiest fusion reaction to achieve is found in a 50–50 mixture of tritium and deuterium.[24] For fusion power experiments this mixture must be held at high temperatures for relatively lengthy times in order to have an efficient reaction. For explosive use, however, the goal is not to produce efficient fusion, but simply provide extra neutrons early in the process.[citation needed] Since a nuclear explosion is supercritical, any extra neutrons will be multiplied by the chain reaction, so even tiny quantities introduced early can have a large effect on the outcome. For this reason, even the relatively low compression pressures and times (in fusion terms) found in the center of a hollow pit warhead are enough to create the desired effect.[citation needed]
In the boosted design, the fusion fuel in gas form is pumped into the pit during arming. This will fuse into helium and release free neutrons soon after fission begins.[citation needed] The neutrons will start a large number of new chain reactions while the pit is still critical or nearly critical. Once the hollow pit is perfected, there is little reason not to boost; deuterium and tritium are easily produced in the small quantities needed, and the technical aspects are trivial.[24]
The concept of fusion-boosted fission was first tested on May 25, 1951, in the Item shot of Operation Greenhouse, Eniwetok, yield 45.5 kilotons.[citation needed]
Boosting reduces diameter in three ways, all the result of faster fission:
- Since the compressed pit does not need to be held together as long, the massive U-238 tamper can be replaced by a light-weight beryllium shell (to reflect escaping neutrons back into the pit). The diameter is reduced.[citation needed]
- The mass of the pit can be reduced by half, without reducing yield. Diameter is reduced again.[citation needed]
- Since the mass of the metal being imploded (tamper plus pit) is reduced, a smaller charge of high explosive is needed, reducing diameter even further.[citation needed]

The first device whose dimensions suggest employment of all these features (two-point, hollow-pit, fusion-boosted implosion) was the Swan device. It had a cylindrical shape with a diameter of 11.6 in (29 cm) and a length of 22.8 in (58 cm).[citation needed]
It was first tested standalone and then as the primary of a two-stage thermonuclear device during Operation Redwing. It was weaponized as the Robin primary and became the first off-the-shelf, multi-use primary, and the prototype for all that followed.[citation needed]

After the success of Swan, 11 or 12 inches (28 or 30 cm) seemed to become the standard diameter of boosted single-stage devices tested during the 1950s.[citation needed] Length was usually twice the diameter, but one such device, which became the W54 warhead, was closer to a sphere, only 15 inches (38 cm) long.
One of the applications of the W54 was the Davy Crockett XM-388 recoilless rifle projectile. It had a dimension of just 11 inches (28 cm), and is shown here in comparison to its Fat Man predecessor (60 inches (150 cm)).
Another benefit of boosting, in addition to making weapons smaller, lighter, and with less fissile material for a given yield, is that it renders weapons immune to predetonation.[citation needed] It was discovered in the mid-1950s that plutonium pits would be particularly susceptible to partial predetonation if exposed to the intense radiation of a nearby nuclear explosion (electronics might also be damaged, but this was a separate problem).[citation needed] RI was a particular problem before effective early warning radar systems because a first strike attack might make retaliatory weapons useless. Boosting reduces the amount of plutonium needed in a weapon to below the quantity which would be vulnerable to this effect.[citation needed]
Two-stage thermonuclear

Pure fission or fusion-boosted fission weapons can be made to yield hundreds of kilotons, at great expense in fissile material and tritium, but by far the most efficient way to increase nuclear weapon yield beyond ten or so kilotons is to add a second independent stage, called a secondary.[citation needed]
In the 1940s, bomb designers at Los Alamos thought the secondary would be a canister of deuterium in liquefied or hydride form. The fusion reaction would be D-D, harder to achieve than D-T, but more affordable. A fission bomb at one end would shock-compress and heat the near end, and fusion would propagate through the canister to the far end. Mathematical simulations showed it would not work, even with large amounts of expensive tritium added.[citation needed]
The entire fusion fuel canister would need to be enveloped by fission energy, to both compress and heat it, as with the booster charge in a boosted primary. The design breakthrough came in January 1951, when Edward Teller and Stanislaw Ulam invented radiation implosion – for nearly three decades known publicly only as the Teller-Ulam H-bomb secret.[25][26]
The concept of radiation implosion was first tested on May 9, 1951, in the George shot of Operation Greenhouse, Eniwetok, yield 225 kilotons. The first full test was on November 1, 1952, the Mike shot of Operation Ivy, Eniwetok, yield 10.4 megatons.[citation needed]
In radiation implosion, the burst of X-ray energy coming from an exploding primary is captured and contained within an opaque-walled radiation channel which surrounds the nuclear energy components of the secondary. The radiation quickly turns the plastic foam that had been filling the channel into a plasma which is mostly transparent to X-rays, and the radiation is absorbed in the outermost layers of the pusher/tamper surrounding the secondary, which ablates and applies a massive force[27] (much like an inside out rocket engine) causing the fusion fuel capsule to implode much like the pit of the primary. As the secondary implodes a fissile "spark plug" at its center ignites and provides neutrons and heat which enable the lithium deuteride fusion fuel to produce tritium and ignite as well. The fission and fusion chain reactions exchange neutrons with each other and boost the efficiency of both reactions. The greater implosive force, enhanced efficiency of the fissile "spark plug" due to boosting via fusion neutrons, and the fusion explosion itself provide significantly greater explosive yield from the secondary despite often not being much larger than the primary.[citation needed]

- Warhead before firing. The nested spheres at the top are the fission primary; the cylinders below are the fusion secondary device.
- Fission primary's explosives have detonated and collapsed the primary's fissile pit.
- The primary's fission reaction has run to completion, and the primary is now at several million degrees and radiating gamma and hard X-rays, heating up the inside of the hohlraum, the shield, and the secondary's tamper.
- The primary's reaction is over and it has expanded. The surface of the pusher for the secondary is now so hot that it is also ablating or expanding away, pushing the rest of the secondary (tamper, fusion fuel, and fissile spark plug) inward. The spark plug starts to fission. Not depicted: the radiation case is also ablating and expanding outward (omitted for clarity of diagram).
- The secondary's fuel has started the fusion reaction and shortly will burn up. A fireball starts to form.
For example, for the Redwing Mohawk test on July 3, 1956, a secondary called the Flute was attached to the Swan primary. The Flute was 15 inches (38 cm) in diameter and 23.4 inches (59 cm) long, about the size of the Swan. But it weighed ten times as much and yielded 24 times as much energy (355 kilotons, vs 15 kilotons).[citation needed]
Equally important, the active ingredients in the Flute probably cost no more than those in the Swan. Most of the fission came from cheap U-238, and the tritium was manufactured in place during the explosion. Only the spark plug at the axis of the secondary needed to be fissile.[citation needed]
A spherical secondary can achieve higher implosion densities than a cylindrical secondary, because spherical implosion pushes in from all directions toward the same spot. However, in warheads yielding more than one megaton, the diameter of a spherical secondary would be too large for most applications. A cylindrical secondary is necessary in such cases. The small, cone-shaped re-entry vehicles in multiple-warhead ballistic missiles after 1970 tended to have warheads with spherical secondaries, and yields of a few hundred kilotons.[citation needed]
As with boosting, the advantages of the two-stage thermonuclear design are so great that there is little incentive not to use it, once a nation has mastered the technology.[citation needed]
In engineering terms, radiation implosion allows for the exploitation of several known features of nuclear bomb materials which heretofore had eluded practical application. For example:
- The optimal way to store deuterium in a reasonably dense state is to chemically bond it with lithium, as lithium deuteride. But the lithium-6 isotope is also the raw material for tritium production, and an exploding bomb is a nuclear reactor. Radiation implosion will hold everything together long enough to permit the complete conversion of lithium-6 into tritium, while the bomb explodes. So the bonding agent for deuterium permits use of the D-T fusion reaction without any pre-manufactured tritium being stored in the secondary. The tritium production constraint disappears.[citation needed]
- For the secondary to be imploded by the hot, radiation-induced plasma surrounding it, it must remain cool for the first microsecond, i.e., it must be encased in a massive radiation (heat) shield. The shield's massiveness allows it to double as a tamper, adding momentum and duration to the implosion. No material is better suited for both of these jobs than ordinary, cheap uranium-238, which also happens to undergo fission when struck by the neutrons produced by D-T fusion. This casing, called the pusher, thus has three jobs: to keep the secondary cool; to hold it, inertially, in a highly compressed state; and, finally, to serve as the chief energy source for the entire bomb. The consumable pusher makes the bomb more a uranium fission bomb than a hydrogen fusion bomb. Insiders never used the term "hydrogen bomb".[28]
- Finally, the heat for fusion ignition comes not from the primary but from a second fission bomb called the spark plug, embedded in the heart of the secondary. The implosion of the secondary implodes this spark plug, detonating it and igniting fusion in the material around it, but the spark plug then continues to fission in the neutron-rich environment until it is fully consumed, adding significantly to the yield.[29]
In the ensuing fifty years, no one has come up with a more efficient way to build a thermonuclear bomb. It is the design of choice for the United States, Russia, the United Kingdom, China, and France, the five thermonuclear powers. On 3 September 2017 North Korea carried out what it reported as its first "two-stage thermo-nuclear weapon" test.[30] According to Dr. Theodore Taylor, after reviewing leaked photographs of disassembled weapons components taken before 1986, Israel possessed boosted weapons and would require supercomputers of that era to advance further toward full two-stage weapons in the megaton range without nuclear test detonations.[31] The other nuclear-armed nations, India and Pakistan, probably have single-stage weapons, possibly boosted.[29]
Interstage
In a two-stage thermonuclear weapon the energy from the primary impacts the secondary. An essential[citation needed] energy transfer modulator called the interstage, between the primary and the secondary, protects the secondary's fusion fuel from heating too quickly, which could cause it to explode in a conventional (and small) heat explosion before the fusion and fission reactions get a chance to start.[citation needed]
There is very little information in the open literature about the mechanism of the interstage.[citation needed] Its first mention in a U.S. government document formally released to the public appears to be a caption in a graphic promoting the Reliable Replacement Warhead Program in 2007. If built, this new design would replace "toxic, brittle material" and "expensive 'special' material" in the interstage.[32] This statement suggests the interstage may contain beryllium to moderate the flux of neutrons from the primary, and perhaps something to absorb and re-radiate the x-rays in a particular manner.[33] There is also some speculation that this interstage material, which may be code-named Fogbank, might be an aerogel, possibly doped with beryllium and/or other substances.[34][35]
The interstage and the secondary are encased together inside a stainless steel membrane to form the canned subassembly (CSA), an arrangement which has never been depicted in any open-source drawing.[36] The most detailed illustration of an interstage shows a British thermonuclear weapon with a cluster of items between its primary and a cylindrical secondary. They are labeled "end-cap and neutron focus lens", "reflector/neutron gun carriage", and "reflector wrap". The origin of the drawing, posted on the internet by Greenpeace, is uncertain, and there is no accompanying explanation.[37]
Specific designs
While every nuclear weapon design falls into one of the above categories, specific designs have occasionally become the subject of news accounts and public discussion, often with incorrect descriptions about how they work and what they do. Examples:
Alarm Clock/Sloika
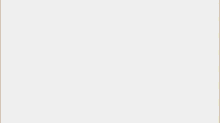
The first effort to exploit the symbiotic relationship between fission and fusion was a 1940s design that mixed fission and fusion fuel in alternating thin layers. As a single-stage device, it would have been a cumbersome application of boosted fission. It first became practical when incorporated into the secondary of a two-stage thermonuclear weapon.[38]
The U.S. name, Alarm Clock, came from Teller: he called it that because it might "wake up the world" to the possibility of the potential of the Super.[39] The Russian name for the same design was more descriptive: Sloika (Russian: Слойка), a layered pastry cake. A single-stage Soviet Sloika was tested on August 12, 1953. No single-stage U.S. version was tested, but the code named Castle Union shot of Operation Castle, April 26, 1954, was a two-stage thermonuclear device code-named Alarm Clock. Its yield, at Bikini, was 6.9 megatons.[citation needed]
Because the Soviet Sloika test used dry lithium-6 deuteride eight months before the first U.S. test to use it (Castle Bravo, March 1, 1954), it was sometimes claimed that the USSR won the H-bomb race, even though the United States tested and developed the first hydrogen bomb: the Ivy Mike H-bomb test. The 1952 U.S. Ivy Mike test used cryogenically cooled liquid deuterium as the fusion fuel in the secondary, and employed the D-D fusion reaction. However, the first Soviet test to use a radiation-imploded secondary, the essential feature of a true H-bomb, was on November 23, 1955, three years after Ivy Mike. In fact, real work on the implosion scheme in the Soviet Union only commenced in the very early part of 1953, several months after the successful testing of Sloika.[citation needed]
Clean bombs

On March 1, 1954, the largest-ever U.S. nuclear test explosion, the 15-megaton Castle Bravo shot of Operation Castle at Bikini Atoll, delivered a promptly lethal dose of fission-product fallout to more than 6,000 square miles (16,000 km2) of Pacific Ocean surface.[40] Radiation injuries to Marshall Islanders and Japanese fishermen made that fact public and revealed the role of fission in hydrogen bombs.
In response to the public alarm over fallout, an effort was made to design a clean multi-megaton weapon, relying almost entirely on fusion. The energy produced by the fissioning of unenriched natural uranium, when used as the tamper material in the secondary and subsequent stages in the Teller-Ulam design, can far exceed the energy released by fusion, as was the case in the Castle Bravo test. Replacing the fissionable material in the tamper with another material is essential to producing a "clean" bomb. In such a device, the tamper no longer contributes energy, so for any given weight, a clean bomb will have less yield. The earliest known incidence of a three-stage device being tested, with the third stage, called the tertiary, being ignited by the secondary, was May 27, 1956, in the Bassoon device. This device was tested in the Zuni shot of Operation Redwing. This shot used non-fissionable tampers; an inert substitute material such as tungsten or lead was used. Its yield was 3.5 megatons, 85% fusion and only 15% fission.[citation needed]
The public records for devices that produced the highest proportion of their yield via fusion reactions are the peaceful nuclear explosions of the 1970s. Others include the 50 megaton Tsar Bomba at 97% fusion,[41] the 9.3 megaton Hardtack Poplar test at 95%,[42] and the 4.5 megaton Redwing Navajo test at 95% fusion.[43]
The most ambitious peaceful application of nuclear explosions was pursued by the USSR with the aim of creating a 112 km long canal between the Pechora river basin and the Kama river basin, about half of which was to be constructed through a series of underground nuclear explosions. It was reported that about 250 nuclear devices might be used to get the final goal. The Taiga test was to demonstrate the feasibility of the project. Three of these "clean" devices of 15 kiloton yield each were placed in separate boreholes spaced about 165 m apart at depths of 127 m. They were simultaneously detonated on March 23, 1971, catapulting radioactive plume into the air that was carried eastward by wind. The resulting trench was around 700 m long and 340 m wide, with an unimpressive depth of just 10–15m.[44] Despite their "clean" nature, the area still exhibits a noticeably higher (albeit mostly harmless) concentration of fission products, the intense neutron bombardment of the soil, the device itself and the support structures also activated their stable elements to create a significant amount of man-made radioactive elements like 60Co. The overall danger posed by the concentration of radioactive elements present at the site created by these three devices is still negligible, but a larger scale project as was envisioned would have had significant consequences both from the fallout of radioactive plume and the radioactive elements created by the neutron bombardment.[45]
On July 19, 1956, AEC Chairman Lewis Strauss said that the Redwing Zuni shot clean bomb test "produced much of importance ... from a humanitarian aspect." However, less than two days after this announcement, the dirty version of Bassoon, called Bassoon Prime, with a uranium-238 tamper in place, was tested on a barge off the coast of Bikini Atoll as the Redwing Tewa shot. The Bassoon Prime produced a 5-megaton yield, of which 87% came from fission. Data obtained from this test, and others, culminated in the eventual deployment of the highest-yielding US nuclear weapon known, and the highest yield-to-weight weapon ever made, a three-stage thermonuclear weapon with a maximum "dirty" yield of 25 megatons, designated as the B41 nuclear bomb, which was to be carried by U.S. Air Force bombers until it was decommissioned; this weapon was never fully tested.[citation needed]
Third generation
First and second generation nuclear weapons release energy as omnidirectional blasts. Third generation[46][47][48] nuclear weapons are experimental special effect warheads and devices that can release energy in a directed manner, some of which were tested during the Cold War but were never deployed. These include:
- Project Prometheus, also known as "Nuclear Shotgun", which would have used a nuclear explosion to accelerate kinetic penetrators against ICBMs.[49]
- Project Excalibur, a nuclear-pumped X-ray laser to destroy ballistic missiles.
- Nuclear shaped charges that focus their energy in particular directions.
- Project Orion explored the use of nuclear explosives for rocket propulsion.
Fourth generation
The idea of "4th-generation" nuclear weapons has been proposed as a possible successor to the examples of weapons designs listed above. These methods tend to revolve around using non-nuclear primaries to set off further fission or fusion reactions. For example, if antimatter were usable and controllable in macroscopic quantities, a reaction between a small amount of antimatter and an equivalent amount of matter could release energy comparable to a small fission weapon, and could in turn be used as the first stage of a very compact thermonuclear weapon. Extremely-powerful lasers could also potentially be used this way, if they could be made powerful-enough, and compact-enough, to be viable as a weapon. Most of these ideas are versions of pure fusion weapons, and share the common property that they involve hitherto unrealized technologies as their "primary" stages.[50]
While many nations have invested significantly in inertial confinement fusion research programs, since the 1970s it has not been considered promising for direct weapons use, but rather as a tool for weapons- and energy-related research that can be used in the absence of full-scale nuclear testing. Whether any nations are aggressively pursuing "4th-generation" weapons is not clear. In many case (as with antimatter) the underlying technology is presently thought to be very far from being viable, and if it was viable would be a powerful weapon in and of itself, outside of a nuclear weapons context, and without providing any significant advantages above existing nuclear weapons designs[51]
Pure fusion weapons
Since the 1950s, the United States and Soviet Union investigated the possibility of releasing significant amounts of nuclear fusion energy without the use of a fission primary. Such "pure fusion weapons" were primarily imagined as low-yield, tactical nuclear weapons whose advantage would be their ability to be used without producing fallout on the scale of weapons that release fission products. In 1998, the United States Department of Energy declassified the following:
(1) Fact that the DOE made a substantial investment in the past to develop a pure fusion weapon Zdroj:https://en.wikipedia.org?pojem=Nuclear_weapon_design
Text je dostupný za podmienok Creative Commons Attribution/Share-Alike License 3.0 Unported; prípadne za ďalších podmienok. Podrobnejšie informácie nájdete na stránke Podmienky použitia.
Antropológia
Aplikované vedy
Bibliometria
Dejiny vedy
Encyklopédie
Filozofia vedy
Forenzné vedy
Humanitné vedy
Knižničná veda
Kryogenika
Kryptológia
Kulturológia
Literárna veda
Medzidisciplinárne oblasti
Metódy kvantitatívnej analýzy
Metavedy
Metodika
Text je dostupný za podmienok Creative
Commons Attribution/Share-Alike License 3.0 Unported; prípadne za ďalších
podmienok.
Podrobnejšie informácie nájdete na stránke Podmienky
použitia.
www.astronomia.sk | www.biologia.sk | www.botanika.sk | www.dejiny.sk | www.economy.sk | www.elektrotechnika.sk | www.estetika.sk | www.farmakologia.sk | www.filozofia.sk | Fyzika | www.futurologia.sk | www.genetika.sk | www.chemia.sk | www.lingvistika.sk | www.politologia.sk | www.psychologia.sk | www.sexuologia.sk | www.sociologia.sk | www.veda.sk I www.zoologia.sk